Playing dice with (cell) fate
- Jack Marsden
- Oct 27, 2020
- 6 min read
Recent research into DNA regulation and its relationship with the fate of cells
The following is based on the article 'Active turnover of DNA methylation during cell fate decisions' by A. Parry, S. Rulands and W. Reik, first published in Nature Reviews Genetics on October 6 2020. Links to that article and other relevant reads can be found in the 'Read more' section below this post.
It’s rare that a piece of research will come out with a eureka moment that answers all of our existing questions in a field of science. And no, this isn’t an article about one of those pieces of research. This is an article about the usual result of research – one question leading to dozens more pointed questions. Whether the topics I talk about today are of interest to you or not, it’s worth noting this pattern of scientific questions being answered not with plain answers, but more questions. The hope is that the questions we are left with at the end are more directed, more specific, and more useful.
As the holder of the genetic code, and therefore the secrets and recipes of living organisms, DNA molecules are heavily regulated. Not by international customs or tax law (not yet anyway), but on a microscopic level by proteins and other molecules in the cell. The mysteries of how, and more importantly, why, DNA molecules are regulated the way they are still unfolding themselves to us. The topic of this article then, is an answer leading to more questions. Today I’ll be writing about one form of DNA regulation, what we’ve learnt about it, and the questions this leads to in future research in the area. That form of regulation is called DNA methylation.
DNA what-ylation?
DNA methylation (DNAme for short) is, in simple terms, the addition of a tiny molecule (a methyl group) to a base of DNA. It’s a form of regulation called epigenetic regulation. Epigenetic is a broad term referring to anything on top of but not including the DNA. For instance, DNA is often found wrapped around proteins called histone proteins. Changes in the shape of those histone proteins and changes in the way the DNA wraps around them are epigenetic modifications, and affect the way the DNA sends out its instructions. In the same way, DNAme has a large effect on the way DNA behaves, and genetic areas with high densities of DNAme (where lots of cytosines are methylated) are less likely to be transcribed and then translated into protein, or will do so at lower rates than the same area without DNAme.
In technical terms, DNAme changes the cytosine base into a base called 5-methyl-cytosine (5mC). This just means that in the carbon ring of the cytosine, the 5’ carbon has a methyl group attached. This is shown below.
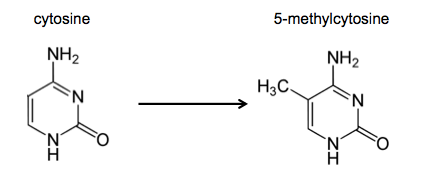
DNAme tends to affect some cytosines more than other cytosines. But which ones? DNAme occurs most frequently in cytosines which can be said to belong to a CpG dinucleotide. In the genetic code, a CpG dinucleotide is any part of the code where the base guanine runs after a cytosine, so the code reads CG. It is estimated that 60-80% of CpG nucleotides contain 5mC instead of regular cytosine. Often, these dinucleotides occur one after the other (so the code reads CGCGCG), and when this occurs over a stretch of 300 or more base pairs, the stretch of DNA is called a CpG island. Somewhat interestingly, DNAme occurs more densely at regions with less CpG dinucleotide repeats, and less densely at regions such as CpG islands. The reason for this will become apparent.
What controls DNA methylation?
Like so many other things in the cell, DNA methylation is controlled by proteins. Proteins are the key molecules associated with regulating the DNA in most instances. Of course, proteins are produced and regulated by the DNA, so after a while it becomes a cycle of DNA producing protein regulating DNA producing protein regulating DNA prod… and it becomes easier to ignore it and let it do its thing.
More specifically, DNAme is initiated by a group of proteins called DNA methyltransferases (DNMTs for short). The proteins DNMT3a, 3b, and 3c are responsible for depositing the methyl groups on cytosines, whereas the protein DNMT1 is responsible for ensuring that the methylation remains during DNA replication. If DNMT1 or its cofactor* UHRF1 become disengaged or aren’t expressed properly, then DNA replication will result in the resulting strands of DNA not being methylated at that point. This is called replication-dependent demethylation, or more simply, passive demethylation.
DNA can also be demethylated more actively, and this is also regulated by proteins. These are called Ten-Eleven Translocation enzymes (TET enzymes for short). When these come into contact with methylated DNA, they alter the 5mC to form the products 5hmC, then 5fC, then 5caC. The type of chemical reaction necessary to move to each of these states is called an oxidation reaction, so 5hmC, 5fC, and 5caC are called the ‘oxidative states’ of cytosine. Both 5fC and 5caC can then be altered by another protein called thymine DNA glycolase (TDG) to return it to its original state as cytosine. Even if this last TDG step doesn’t take place, DNMT1 is usually unable to bind to any of these oxidative states, so they will almost always be demethylated passively when the DNA eventually. Though passive demethylation will always occur, TET enzymes will make this process three times as efficient. TET enzymes always bind more readily at CpG rich areas such as CpG islands, explaining why these regions are less methylated than others.
The DNAme cycle, and why should anybody care anyway?
From these two mechanisms of methylation and demethylation, it is easy to see how a cycle is formed. The cycle is referred to as DNAme turnover. This isn’t surprising, most things in biological systems occur in cycles. So why do we care? This is perhaps getting a bit niche (I mean this whole website is a bit niche, but you’re here so let’s continue), but it’s to do with speed. More specifically, it’s got to do with speed in a particular type of cell – a pluripotent stem cell.
Interlude – What do you want to be when you differentiate?
A pluripotent stem cell is essentially a master cell. It has the potential to become any cell in that organism. Broken down, pluripotency means the potential to be a number of things, and that’s what these cells are capable of. They remain pluripotent for a while, then eventually exit pluripotency by becoming a nerve cell, or a muscle cell, or a leaf cell (OK, so no organism has leaves and muscles, but you get the idea). This exiting of pluripotency is called differentiation. Once a pluripotent stem cell has differentiated, it has now ‘decided’ on a path – becoming a nerve stem cell, a muscle stem, or a leaf stem cell, from which tissues can grow. Now back to the main article.
A key observation about pluripotent stem cells is their methylation patterns – pluripotent cells are relatively extremely demethylated. What isn’t so clear is whether demethylation is a condition necessary to enter the state of pluripotency, or if it’s just a kind of byproduct. When pluripotent stem cells differentiate, methylation goes up to usual cellular rates. But just before that happens, we get DNAme turnover occurring at rapid rates. Like really, really fast. Like a full cycle every 2-3 hours (no but that is actually very very fast trust me I have a website). This speed is brought on by binding various proteins to TET enzymes and DNMTs and making them work more and more efficiently.
Conclusion – what have we really learnt?
The great mystery here is the necessity of this process. It’s unclear whether DNAme turnover is essential to the differentiation of pluripotent stem cells or whether it’s just a byproduct of the swirling maelstrom that is differentiation. If it’s essential, there’s a chance that the rate of DNAme and the oxidation state of the cellular cytosines have an impact on the type of cell the pluripotent stem cell becomes. This would be an example of a physical phenomenon called symmetry breaking, where a huge number of incredibly small fluctuations (in this case, the oxidation states of millions of cytosines) impact a much larger outcome (the nature of the resulting cell).
As I alluded to earlier, this is a fantastic example of an initial question – what is happening, where is it happening, how is it happening – leading to a more substantive question – why is this happening there like that? And other, more pointed questions which can form the basis of future research. Further research will drive us closer to a proper understanding of the importance of DNAme turnover and its role in cell fate: determining the identity of cells, whether genetic mutations affect this if they do, whether that creates disease, and if they do, whether there’s anything we can do to prevent these diseases.
I hope you learnt something new!
Thanks for reading,
Jack
Read more:
https://www.nature.com/articles/s41576-020-00287-8 - original article
Article cover image source: Christoph Bock, Max Planck Institute for Informatics
*Many proteins have cofactors, which can be thought of as helper proteins necessary to complete their function.
Comments